STRUCTURES Blog > Posts > Structured Rings and Gaps: Is This Where We Come From?
Structured Rings and Gaps: Is This Where We Come From?
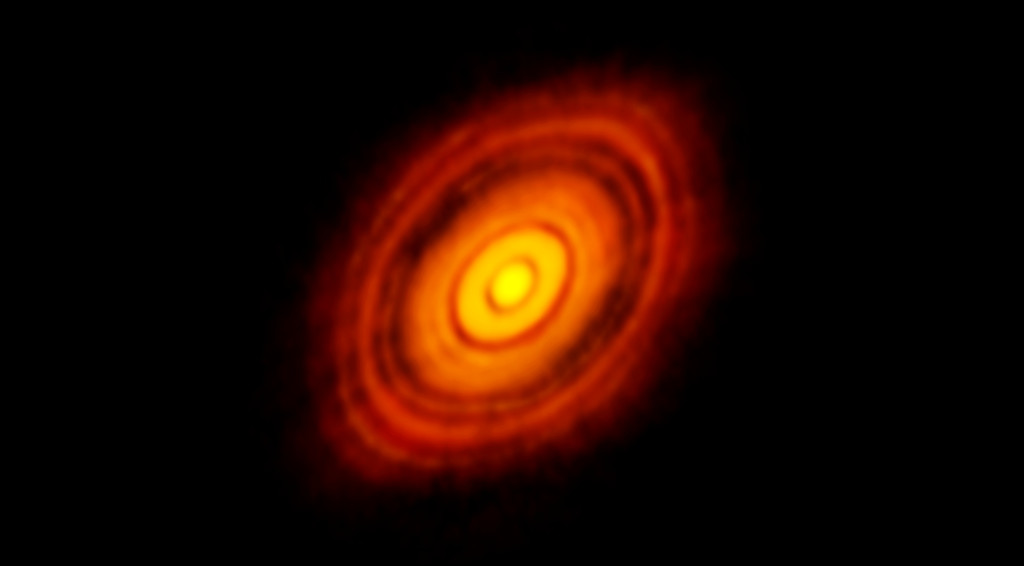
The above image shows beautiful structures such as rings and gaps in an astrophysical object – a truly remarkable image. But what is it? A part of this question is answered easily: it’s an observation of a young star called HL Tau, surrounded by a disk out of gas and dust. But the further part, the question about the rings and gaps and where they come from, is much more difficult to answer. In this article, let me guide you through the story of the disk’s structures and how this image accelerated research in the field of planet formation.
The object in the image is a so-called protoplanetary disk. Such disks form together with their central star, which in turn originates from a collapsing molecular cloud (you can find a more detailed picture of star formation in this blog post). Because everything is dynamic in the universe, it is likely for the molecular cloud to have a slight rotation in the beginning. During the collapse, this rotation becomes stronger, which is due to the same physical principle ice skaters make use of to spin incredibly fast by pulling in their limbs, namely conservation of angular momentum. Because of this principle, the molecular cloud starts to spin faster as it contracts, and material accumulates in a disk. Those disks are also the origin of planets, but let’s hold that thought for a moment and take a closer look at the disks first.
Long before researchers acquired the stunning image of the disk around HL Tau, we knew that protoplanetary disks exist, even though the observational technology was not good enough to resolve the star and disk individually. The strongest indication for the disks’ existence was the spectrum of a young star: it shows an excess of infrared radiation that can not be explained by the star alone. The infrared excess is caused by the disk, which is heated by the star in its centre. Due to the heat, the disk sends out thermal radiation and contributes to the star’s spectrum. On the other hand, older stars have not been observed to display this excess in infrared radiation, indicating that the lifetime of a disk is much shorter than that of a star. Indeed, scientific studies found that disks live a thousand to ten thousand times shorter than their stars.
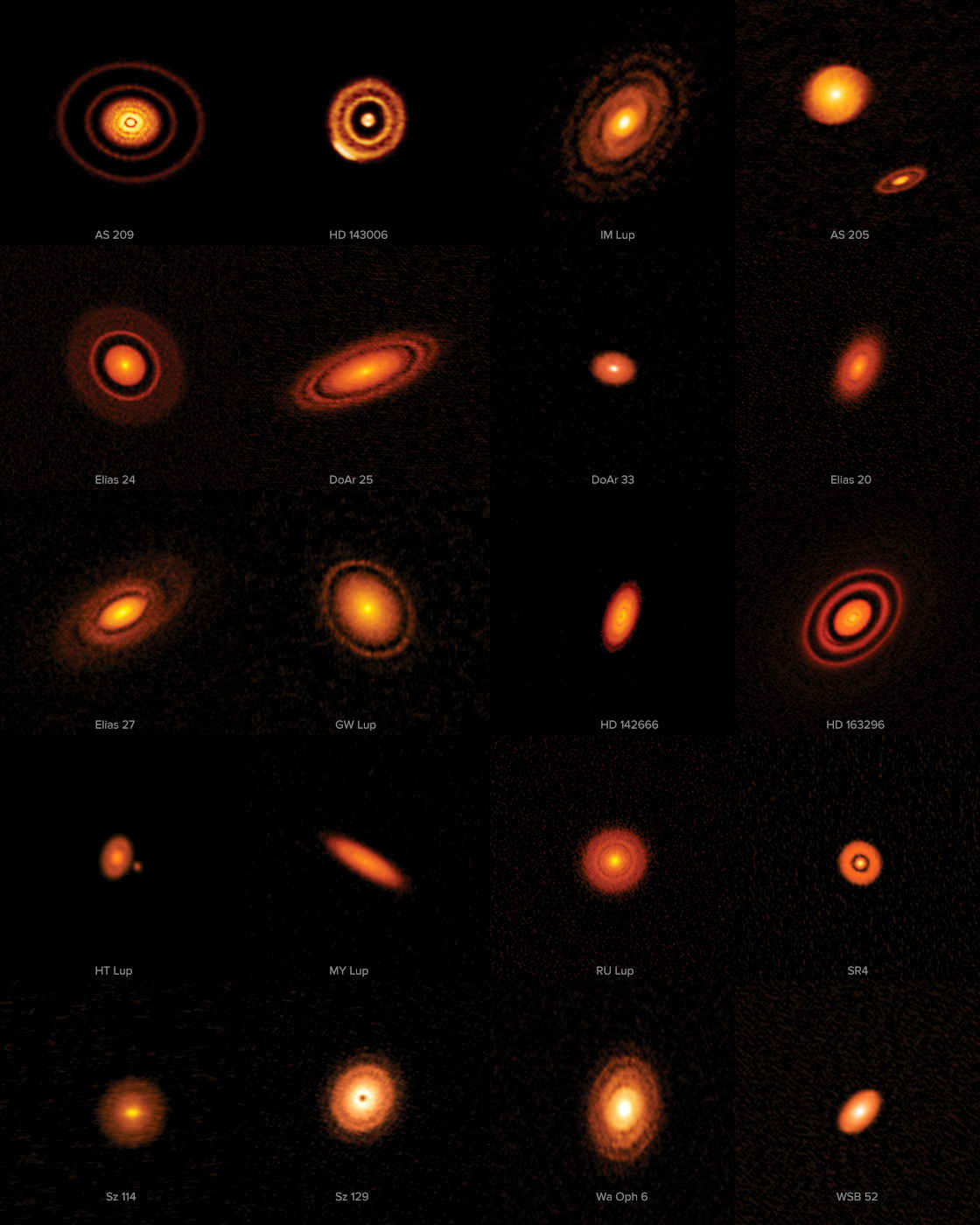
Then, in 2014 (not even ten years ago!), high resolution observations finally revealed HL Tau’s disk as the first well resolved protoplanetary disk. Although observations before that time were able to show that disks exist, the images lacked resolution. HL Tau amazed the research community with its striking details: sharp rings and gaps that had not been observed before. Ever since then, more and more observations of detailed, highly resolved protoplanetary disks have become available. And all of them look different! There is a huge variety of structures: rings, gaps, spirals, arcs, shadows, and more. Fig. 1 shows the results of a disk survey called DSHARP.
A prominent question when looking at these observations is: where do these structures come from? The answer to that is still ongoing research, and there are several possibilities to explain the structures. One of the most common explanations is: a planet. When a planet forms in a disk, it interacts with its gas and dust, stirring everything up. If the planet is big enough, it creates a gap by pushing most of the material to the sides and additionally consuming some of it. Because in such a gap, there is only little material left, it appears dark in observations. At the edges of a gap, some of the material pushed aside by the planet can pile up and appear as bright rings. In addition to that, every planet causes spiral structures in a disk. Such spiral structures are indeed observed in some disks, but as they might be weak, they are not necessarily seen in every disk with a planet.
Advanced: Why Do Planets Cause Spiral Structure in Protoplanetary Disks?
A planet interacts with the material in the disk. Its mere presence causes density perturbations that travel as waves through the disk. These waves behave like sound waves, meaning they are compression waves. The waves produce overdensities in some places, which look brighter as more material is present in these locations.

To understand why the overdensities obtain a spiral shape, we need to look at the orbital velocity of the material in the disk. Any particle, whether it is gas, dust, or a planet, has to maintain a force balance in order to stay on a stable orbit around the star. This means that the centrifugal force $F_{CF}$, that the particle experiences due to its orbital velocity, has to be equal to the gravitational force $F_G$ from the star. $$ {F}_{G} = \frac{G {M}_{\star} {M}_{p}}{r²} = {M}_{p} \frac{v²}{r} = {F}_{CF}. $$ Here, $G$ is the gravitational constant, $M_{\star}$ is the mass of the star, $M_p$ the mass of the particle, $r$ the distance between particle and star, and $v$ the particle’s velocity.
If we solve this equation for velocity, we find $$ v = \sqrt{\frac{GM_{\star}}r}, $$ which means that the farther away from the star a particle is, the slower is its orbital velocity.
Note: This is one of the most fundamental concepts in orbital dynamics and is called Kepler velocity or Keplerian motion.
Returning to the density waves caused by a planet, we now know that the velocity of the material is faster in the region between the star and the planet (“inside”) and slower on the other side (“outside” of the planet). Therefore, when density waves are launched at the planet’s location, they get sheared: on the inside, the fast material will pull and spread the overdensity in front of the planet, while on the outside it is dragged behind. This is how the overdensities smear out into a spiral shape as shown in Fig. 2.
Although planets are not the only possibility to explain the structures in protoplanetary disks, they certainly are of great interest to our society. We as humans naturally wonder where we come from, and investigating the early evolution of stars and planetary systems brings us one step closer to answering the question. However, the process of planet formation is still not completely understood and there are many unanswered physical challenges. So let’s dive into the current state of research.
The starting point for planet formation is a protoplanetary disk containing lots of dust particles. They are mainly silicates and have actually similar properties to dust on Earth: in a room that hasn’t been vacuumed in a while, dust can collect and form dust bunnies. Similar dust bunnies form in a protoplanetary disk and can then stick together. The surrounding pressure in the disk can compress them into small pebble stones. But at this point, we already reach one of the big challenges of understanding planet formation: how can these pebbles grow further? They do not stick together: imagine you throw a pebble stone at another one, they would bounce off each other, right? If you throw them hard enough, they might even fragment and break into small dust particles again. However, we know that planet formation must be possible as Earth and thousands of other planets are the proof for that. One idea to tackle this challenge is to consider ways to make pebbles stickier. This could, for example, be a thin ice coat around them. However, this step is still part of the current research in planet formation.
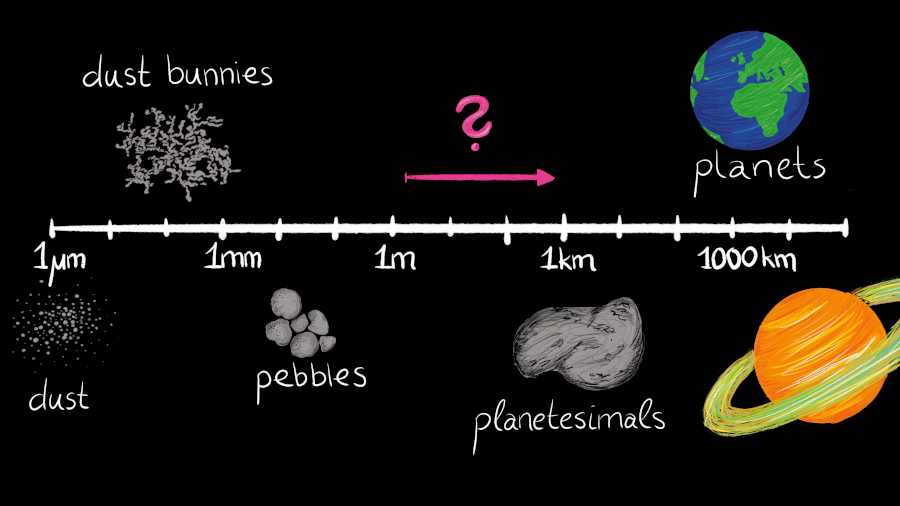
Once a boulder has reached a size of about one kilometre, the process of planet formation is much easier to explain. Such a large boulder, often called planetesimal, already has enough mass to attract and consume the small pebbles and dust particles due to its gravity. This way, planetesimals can grow very efficiently. If one collects more than a hundred times the mass of Earth, it can even attract a huge part of the disk’s gas and a gas giant like Jupiter or Saturn is born. But smaller rocks can also end up as planets, the so-called rocky planets like Earth or Mars.
To answer the title question of this blog post: in our current picture of planet formation, we believe that the Solar System formed in a protoplanetary disk. Therefore, studying those disks as the birthplaces of planets, helps us to progressively answer the question of where we come from. And although we already know a lot about protoplanetary disks, there is still much to learn – an ongoing journey in research.
Tags:
Astrophysics
Fluids
Physics
Structure Formation
Planets
Simulations