STRUCTURES Blog > Posts > Leavitt's Law – or: The Woman Who Changed Astronomy
Leavitt's Law – or: The Woman Who Changed Astronomy
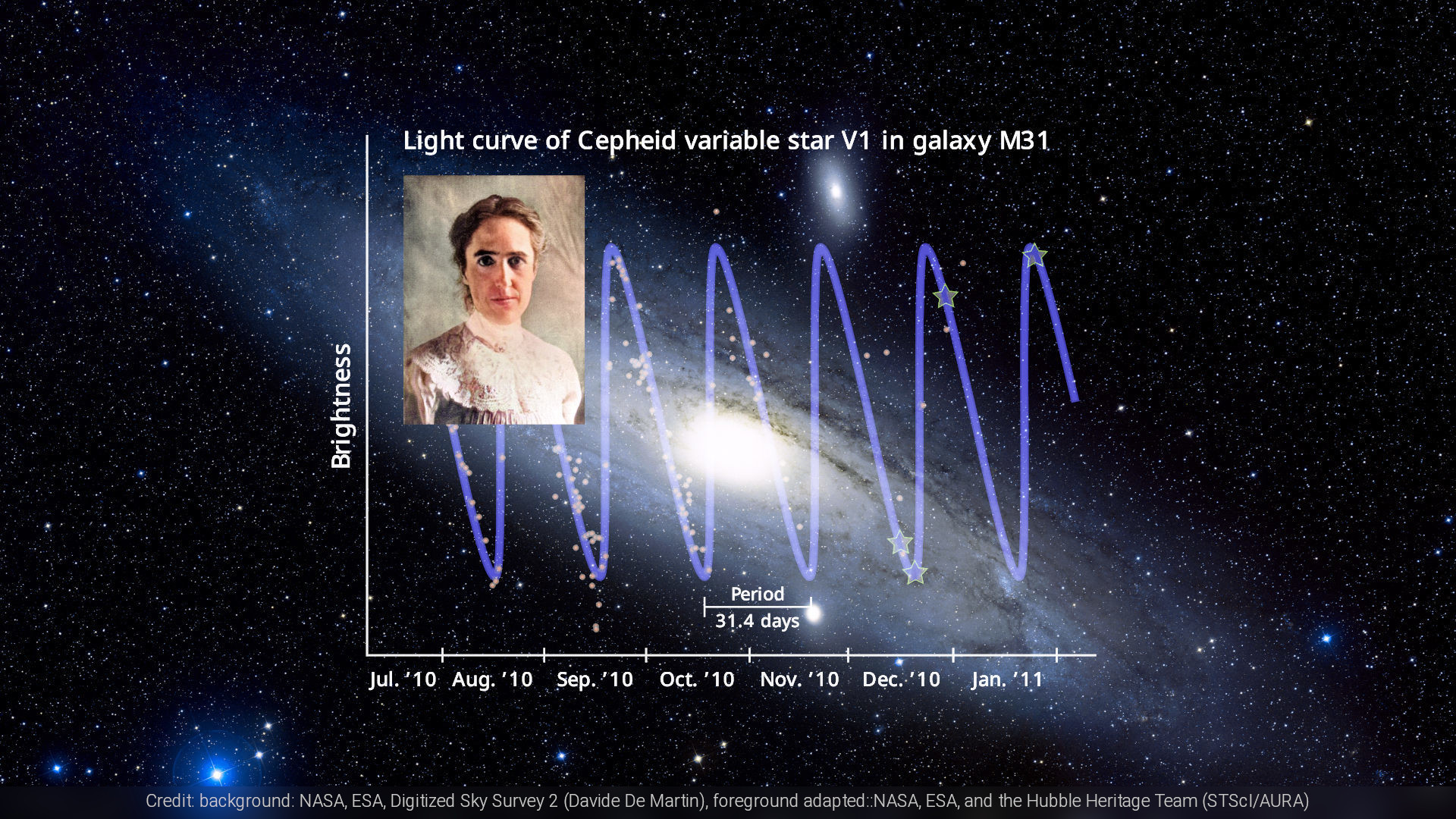
A century ago, the pioneering work of astronomer Henrietta Swan Leavitt revolutionized astronomy. Her work was crucial and changed our picture of the universe. What does this have to do with the mysterious property of pulsating variable stars?
“Space is big. You just won’t believe how vastly, hugely, mind-bogglingly big it is. I mean, you may think it’s a long way down the road to the chemist’s, but that’s just peanuts to space.”
– Douglas Adams, The Hitchhiker’s Guide to the Galaxy
How big is the cosmos, and what is our role in it? Questions like these have intrigued humanity for millennia. As we have peeked ever deeper into the cosmos, our understanding has improved drastically. Five centuries ago we removed Earth from the centre of the cosmos and placed the Sun at the heart of our planetary system. Later on, it was recognized that we are surrounded by billions of other stars like our Sun – even in the relatively small fragment of the spiral arm we inhabit within the Milky Way galaxy. In turn, the Milky Way itself is just one of at several hundred billion galaxies in the observable universe at least. From a cosmological point of view, entire galaxies – these enormous entities whose vastness already challenges human imagination – appear as tiny as molecules in a vast, possibly boundless, ocean. This is not just a metaphor. On very large scales, the cosmos can be described with the same equations that model the behaviour of fluids, with galaxies being vanishingly small point particles.
The insight that our Milky Way is just one of a myriad of galaxies in the observable universe is very recent. It is difficult to imagine today that only a century ago, we did not even know about the existence of other galaxies. Until 1924 it was unclear whether the cosmos was bigger than the Milky Way. Although astronomers had seen and studied the light from – what we know today – other galaxies, their distances were unknown. Thus astronomers could not be sure whether these objects – at the time dubbed spiral nebulae – were tiny structures within the Milky Way or similarly huge objects outside, far beyond our galaxy. Both scenarios seemed possible, highlighting the grave importance (and the challenge) of measuring distances in astronomy. Knowing those is crucial for understanding and interpreting what we see in the sky and to find our place in the cosmos.
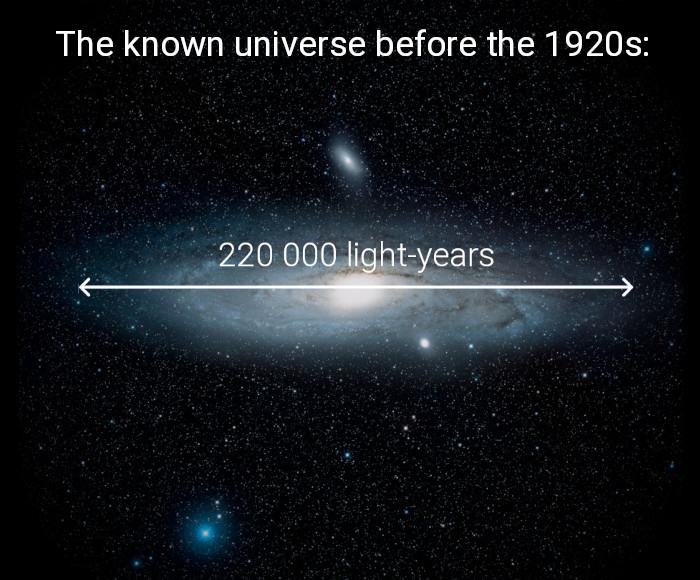
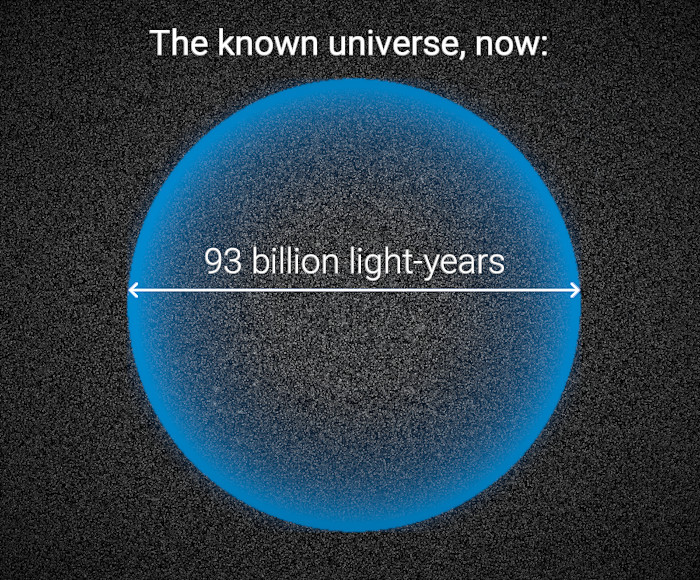
The Issue of Measuring Large Distances in Astronomy
Until the 1910s astronomers had few methods to measure cosmic distances. A key method was the parallax effect, which is quickly explained through an everyday experience. You can observe this effect yourself by holding your thumb up at arms length, closing your left eye and aligning your thumb with an object across the room. Now, switch between opening your right eye and your left eye, and you’ll notice your thumb’s position apparently jumping between two positions in relation to the more distant object. Bringing your thumb closer to your eye, the shift will be bigger. This is the essence of parallax.
The same effect can be used to measure distances in the nearby cosmos: as our Earth moves around the Sun, we can see nearby stars moving apparently larger distances across the sky during the year than distant stars – and measuring this apparent shift allows us to conclude exactly how distant they are. This simple and elegant method, however, works only up to relatively small distances. Beyond a certain distance of another star, the apparent movement across the sky becomes so tiny that the effect can no longer be resolved. It was in no way possible to use it for determining how far spiral nebulae were away. Another idea was needed.
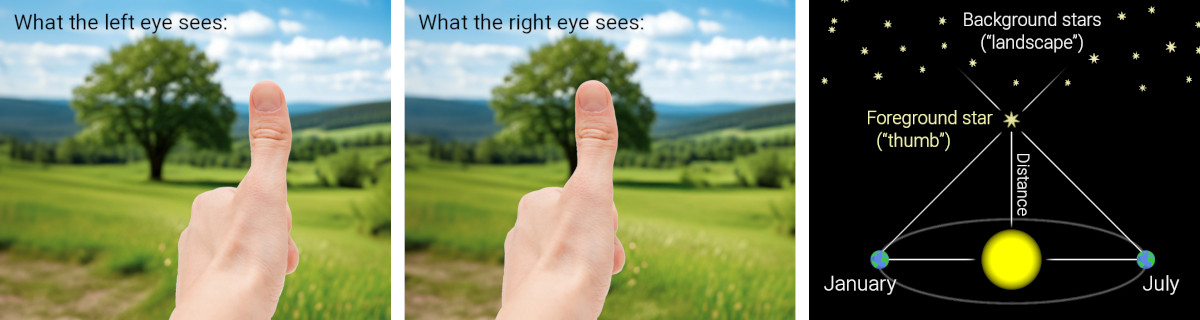
A Revolution in Distance Measurements: Leavitt’s Law for Cepheids
The big breakthrough in mapping distances was accomplished in 1912 by Henrietta Swan Leavitt, an astronomer at Harvard College Observatory.
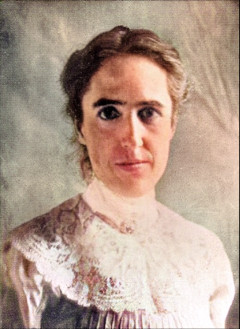
(1868-1921)
Astronomy pioneer
Born in Lancaster, Massachusetts in 1868, Leavitt displayed an early fascination with stars, which led her to pursue her passion for an astronomy career. In 1893, she enrolled at Radcliffe College, where she excelled in her studies and developed a keen interest in variable stars. Despite the rigorous education she received, ranging from classical Greek to fine arts and philosophy, as well as analytical geometry and calculus, she faced limited opportunities in the male-dominated scientific environment of her time. After graduating, when she began working at Harvard College Observatory in 1893, her role was not that of an astronomer, but what was then called “computer,” a term used to describe women hired to perform mathematical calculations and analysis of astronomical data. Leavitt’s meticulous attention to detail, innovative thinking, and relentless pursuit of knowledge set her apart, earning her the respect and admiration of fellow astronomers. In 1921, she was promoted to head of the stellar photometry department.
Leavitt had been examining photographic plates of the Small Magellanic Cloud, a small galaxy orbiting the Milky Way, as we know today. She focused her attention on an interesting and peculiar class of stars known as Cepheid variables. What makes these stars special is that their brightness varies – it pulsates in a regular pattern (Note: pulsation is not to be confused with pulsars!). Cepheids are not the only family of variable stars. In general, brightness variations can have several different reasons: sometimes stars have companions eclipsing them, so that a part of their light gets blocked. In other cases, starspots may cause temporary dimming. Cepheids, however, are variable because they change their actual brightness over time. They rhythmically get brighter and dimmer in a highly regular pattern, which happens over timescales of only days or weeks. Typically, their brightness rises rapidly to a maximum, followed by a slower decrease.
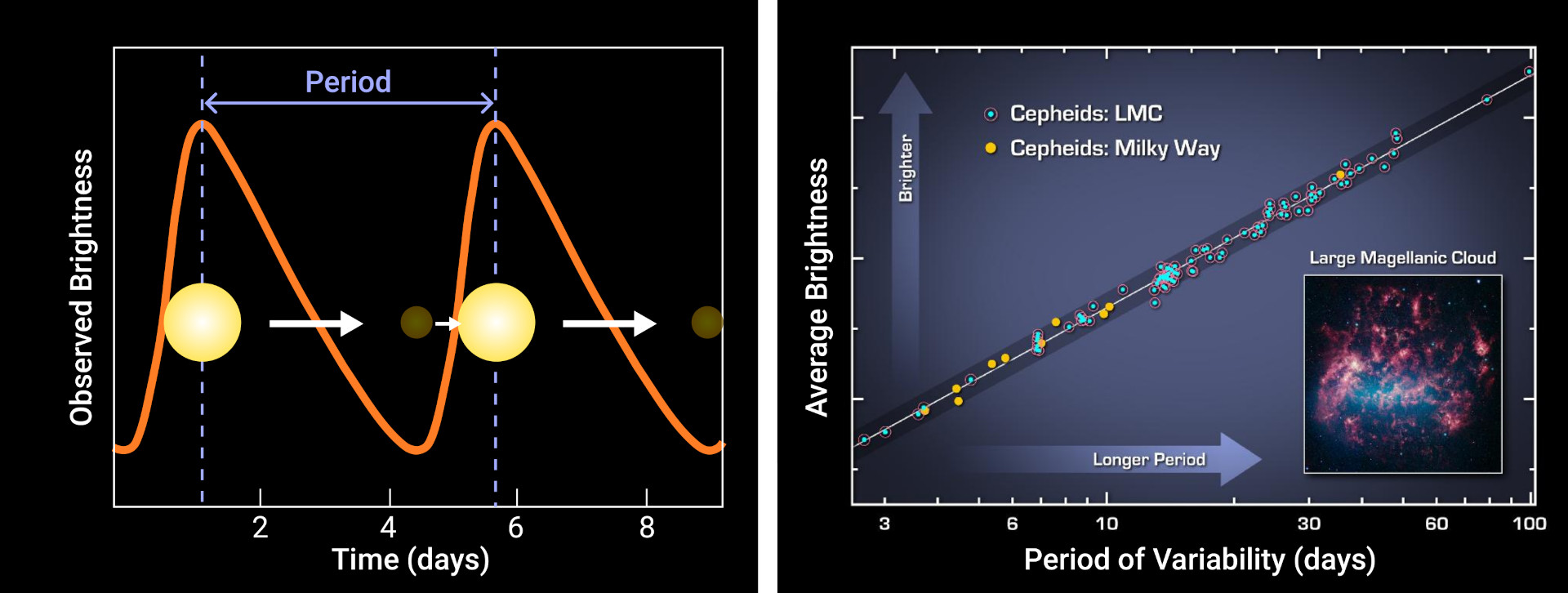
Leavitt’s observational studies of Cepheids revealed an intriguing phenomenon. By 1912, Leavitt had identified 25 such Cepheids and made a groundbreaking discovery. Through meticulous analysis, Leavitt spotted a correlation between Cepheids’ pulsation periods and their brightness. The brighter the star (on average), the longer the interval between two consecutive brightness maxima. Soon, Leavitt had gathered a big enough sample of stars (all located in the small Magellanic Cloud, thus at roughly the same distance) to show evidence for what became known as the period-luminosity relationship – or simply Leavitt’s law.
Leavitt knew that being able to know stars’ true brightness, also called intrinsic luminosity, was like a holy grail in astronomy. A star’s intrinsic brightness is not directly observable because we can only measure its apparent brightness as seen from Earth. Light sources appear dimmer the further they are away, as the light is radiated into each direction, therefore diluting. Purely due to geometric reasons, the apparent brightness has to drop quadratically with distance. This meant that if there was any possibility to determine a star’s intrinsic brightness, we could immediately determine its distance by comparing apparent to intrinsic brightness and applying the quadratic law. Leavitt’s law exactly allows us to do this. Very elegantly, it links the quantity we cannot directly observe – the intrinsic brightness – to a quantity we can very easily observe: the pulsation period, given by the interval between brightness maxima of the Cepheids. The spectacular aspect of Leavitt’s discovery is that it provides us with a simple, yet powerful technique for measuring distances, known as the use of standard candles.
Why Do Cepheids Pulsate?
The Most Important Star in Cosmology
With this knowledge, observing the pulsation period of Cepheid stars allowed astronomers to measure the distance to any place where they could see a Cepheid – be it distant star clusters or nearby galaxies. With this new tool at hand, astronomers could not only measure the size of the Milky Way more precisely than ever before. Quite importantly, the puzzle about the size of the cosmos could finally be settled.
One hundred years ago, in 1924, American astronomer Edwin Hubble used Leavitt’s technique to measure the distance to the Andromeda nebula. He had spotted a Cepheid star in Andromeda that received the name “V1”. In a certain sense, V1 would turn out to become the most important star for cosmology at the time: despite some serious observational uncertainties, Hubble’s distance measurement to V1 revealed that Andromeda could not be part of the Milky Way, but had to be a galaxy of its own. Today, we know that our neighbouring galaxy Andromeda is located about 2.5 million light-years away. The insight that spiral nebulae were actually far-distant galaxies spread over a vast cosmos meant that our Milky Way galaxy was just one in a myriad of galaxies. Virtually overnight, our idea of the size of the universe grew by huge orders of magnitude.
But hold on: this was not the only revolutionary insight in cosmology within a short time. Only three years later, Belgian priest and theoretical physicist Georges Lemaître formulated the famous Hubble-Lemaître law, describing a relation between galaxy velocities and distances in an expanding universe. In 1929, Hubble and Milton Humason independently re-discovered this law and provided empirical evidence by combining Cepheid distances to several galaxies with Vesto Slipher’s measurements of galaxy velocities, confirming the theories of Georges Lemaître. These and follow-up works marked a crucial cornerstone for the development of the big bang cosmological model. Even to this day, Cepheids serve as indispensable segments in what cosmologists call the “distance ladder”, a chain of tools in which each distance measurement method calibrates the subsequent one applied to farther objects.
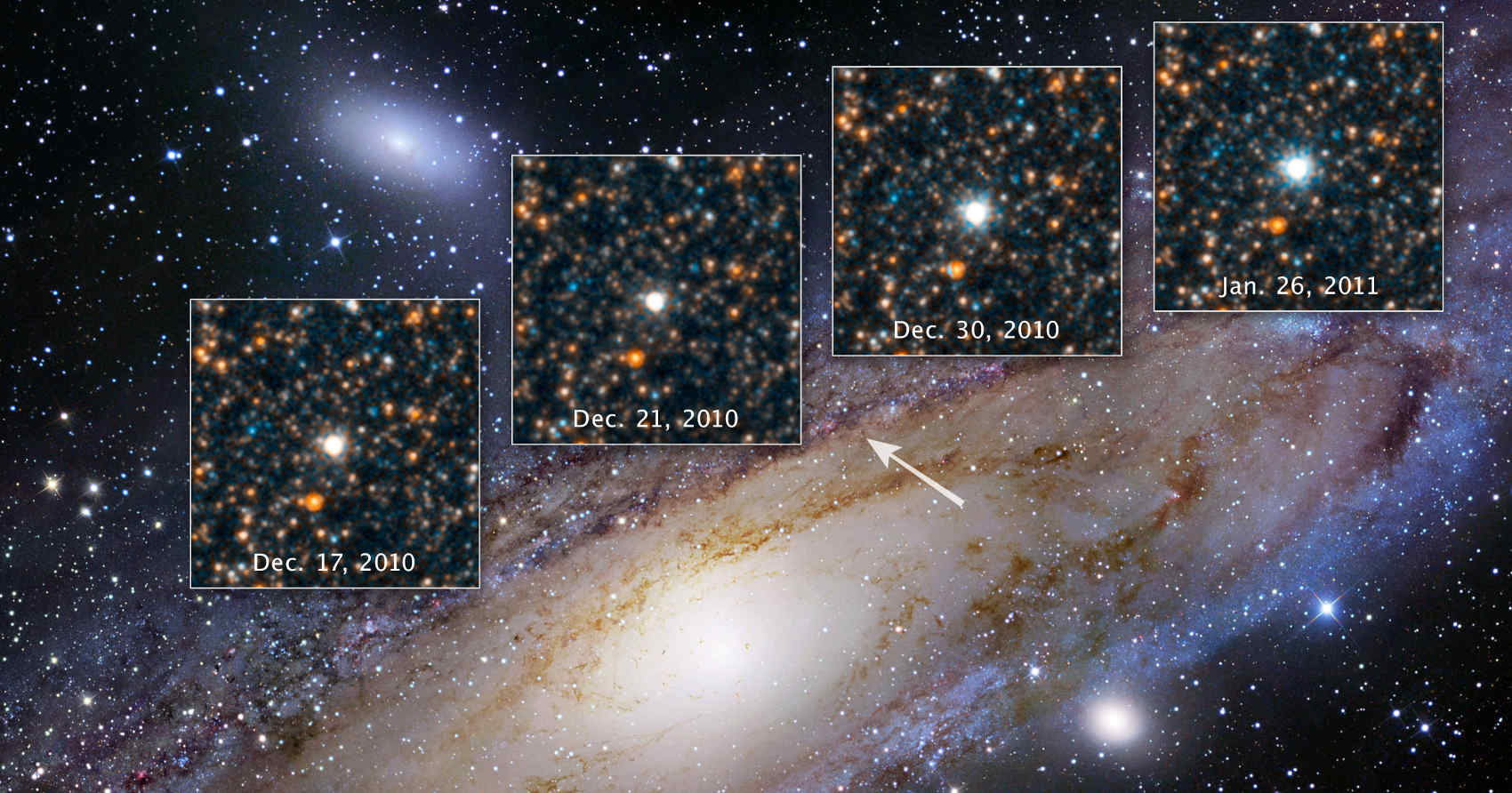
Henrietta Leavitt – a Female Role Model in Science
The life and work of Henrietta Leavitt is impressive. Leavitt was a pioneer who pursued her career in a science system that was everything else than welcoming towards women, and who always lived in the shadow of men like Edwin Hubble. Having been appointed as a permanent staff member at Harvard Observatory in 1902, Leavitt rose to head the department of photographic stellar photometry “by her scientific ability and intense application” (quote from her biography on the American Association of Variable Star Observers, AAVSO). At that time, this was highly extraordinary. Leavitt was also an encourager and instructor: she was deeply committed to advocating for “a place for women in the professions,” actively encouraging young women to pursue scientific careers. The implications of Leavitt’s work were immense. Despite her monumental contributions, Henrietta Leavitt remains relatively unknown compared to her male contemporaries. Not only to the public, but also to the next generations of rising astronomers. While an asteroid and a moon crater bear her name, the true measure of her legacy lies in the scientific discoveries and advancements that have reshaped our understanding of the universe. Sir James Jeans once referred to Cepheids as “lighthouse” stars, not only to describe their pulsation, but in recognition of the role they have been playing as guides to stellar astronomy. Practically, Leavitt’s law became a yardstick for plumbing the depths of space. It provided a representation of the galaxy to scale much as Kepler’s laws had once done for the solar system.
Why is it that everyone of us knows the name of Kepler but few know Henrietta Leavitt?
As you might have guessed, this is not the only example where the achievements of women in science are underemphasized compared to similar achievements by their male counterparts. This is known as the Matilda effect. Rosalind Franklin’s contribution to the discovery of the DNA structure was not properly credited as James Watson and Francis Crick received the Nobel Prize in 1962. Lise Meitner and Otto Hahn discovered nuclear fission together - however, Hahn received the Nobel Prize in Physics for it alone. Statistical evidence for the Matilda effect has been found in scientific studies (e.g. Knobloch-Westerwick, Glynn, and Huge, 2013, which investigates gender bias in perceptions of research quality and collaboration interest).
But what are the consequences of the Matilda effect? Discouragement of the scientist who is directly involved. Less recognition of the scientific result itself, slowing follow-up science by the community. And not to speak of the complex consequences of missing female role models. This is bad for science, bad for the people involved and thus bad for society.
No doubt had – or in some fields still has – science been a highly male-dominated place in which women were denied equal opportunities, let alone support and encouragement. So there are more stories of successful male than female scientists to tell. Throughout history, countless generations of young boys have undoubtedly been inspired by the lives and discoveries of towering figures like Sir Isaac Newton, Albert Einstein, or Edwin Hubble, to pursue careers in science. But on top of that, the way how we tell science bears the potential of drawing an even worse picture and thus reproducing these problems. Imagine the following situation today: there is this successful female scientist being celebrated not only for her scientific accomplishments, but also as a breakthrough for equality in a male dominated area. While this woman for sure deserves most credit for her successes and the obstacles she had to overcome, the community itself should put their triumph for “finally bringing a woman into a successful position” into perspective: there have always been successful women! This is neither a new invention nor is this a recent achievement. Underemphasizing the achievements by previous successful women brings the risk of overestimating our current accomplishments to make science a more diverse place which in turn brings the risk to make actually less real progress.
When it comes to science history it determines how we narrate science e.g. in text books or lectures and how we rate our situation today. Habits and societal biases within the scientific community may play roles in shaping these narratives and are passed on from one generation of scientists to the next. Even if we are not aware of it! This is where another problematic effect comes in: the tendency for historical narratives to persist over time, even when they are inaccurate or incomplete – an effect the authors of this article have experienced multiple times in their career, from their student years up to becoming researchers and teachers in astrophysics. We thus may perpetuate certain selective or interpretational biases of these narratives. This already starts with language. An example concerns Georges Lemaître and Edwin Hubble themselves: for decades, the redshift-distance relation in an expanding universe has simply been referred to as “Hubble’s law”, overlooking Georges Lemaître’s earlier formulation. Only in 2018 did the International Astronomical Union rectify this oversight by renaming it the “Hubble-Lemaître law,” acknowledging Lemaître’s seminal contribution. However, this recognition came late, and the enduring perception that Hubble alone discovered this law persists among generations of astronomers.
So what can we do about that today, apart from renaming scientific laws and writing blog articles about successful women in science? First of all the good news is that not only sexists are biased - and YES that is a good thing, because not everyone who is biased is sexist! In fact, we are of course all biased in one way or another, because we live in a world where tales are told, and recurring schemes are – well - recurrent, leading to certain narratives we were getting used to, typically without even consciously knowing. These may lead to us being more open to a narrative in which the great hero or discoverer is male – be it just because those were told to us more often and have therefore become our (unconscious) habits. To make a difference, we need to understand these subtle, often unconscious effects at play. And here comes the next good thing: being aware that such mechanisms exist – in our heads, in books, and throughout the scientific community – even if we have the best intentions – enables us to overcome these biases and act accordingly.
When looking at the past, the social and cultural context of a decade, as well as its stereotypes, biases and hypocrisies are inseparably reflected by textbooks from this time – whether the authors wanted or not. Just as the historic context needs to be always taken into account when reading such sources, we might have to learn to be more critical towards “common belief” and “textbook knowledge”, but also towards ourselves. After all, this affects how we judge scientists and the importance of their research today as well, with consequences for science as a whole. Thus, when we look into the future, bear in mind that it matters how a story is told and who the hero or the heroine is.
IMPORTANT NOTE / DISCLAIMER:
The authors want to stress that this text reflects their personal understanding, opinions, and very subjective experiences. This text is by no means meant to deliver an objective or complete picture on the highly complex topic of women and other underrepresented groups in science. The examples that are presented here and the conclusions that are drawn are not meant to condemn or to offend but to motivate critical thinking and self-reflection such that science not only becomes an even better place for everyone but also grows further in the best possible ways.
Little Dictionary for Non-Astrophysicists
Observable Universe (German: Beobachtbares Universum)
Since our universe has a finite age - approximately 13.7 billion years - and the speed of light is finite - approximately 300 000 000 m/s, we can only observe a finite volume of the universe. This portion is dubbed “the observable universe”. Whether space extends to infinity, i.e. whether the universe is infinitely large, is something we cannot observe directly, and we do not know up to now.
Parallax Effect (German: Parallaxeneffekt)
With today’s telescopes the parallax effect, or stellar parallax method, can be used reliably for distance measurements up to 5-10 kilo parsecs or 15,000-30,000 light years. A hundred years ago, parallax measurements could be used to determine the distance of stars within a few hunderd light years from earth. That is pretty small compared to the size of our Milky Way galaxy, which has a diameter of more than 100,000 light years!
Luminosity (German: Leuchtkraft)
Luminosity (whether observed or absolute), refers to the total amount of light emitted by a celestial object, either as perceived from Earth or as if viewed from a standard distance, respectively.
Standard candle (German: Standardkerze)
An astronomical object whose intrinsic brightness is known, allowing astronomers to use its observed brightness to determine distances to other objects.
Nuclear fission (German: Kernspaltung)
The process in which the nucleus of an atom splits into two or more smaller nuclei. In this process, a significant amount of energy is released, which is the energy that fuels nuclear power plants.
Tags:
Astrophysics
Cosmology
Women in Science
Stars
Galaxies
Physics
Correlations